Food & Drinks: Soon to be High Tech Goods (The Century of Biology: Part II.III)
Over the next several decades, world food demand is expected to rise by 50%, and demand for animal products by at least 70%. Said another way, every year for decades, we need to feed ~60 million more people. And, as the world becomes wealthier, its citizens demand more meat. Just look at China below. However, we already have 820 million people with insufficient access to food and a climate crisis when food production generates a quarter of GHG emissions and consumes roughly half of the world’s habitable land. Adding another 2 billion people to this planet by 2050 and a significant portion of the world rising to middle income and above would make our climate objectives impossible and would flatten more forests without fundamentally rethinking how we make food.
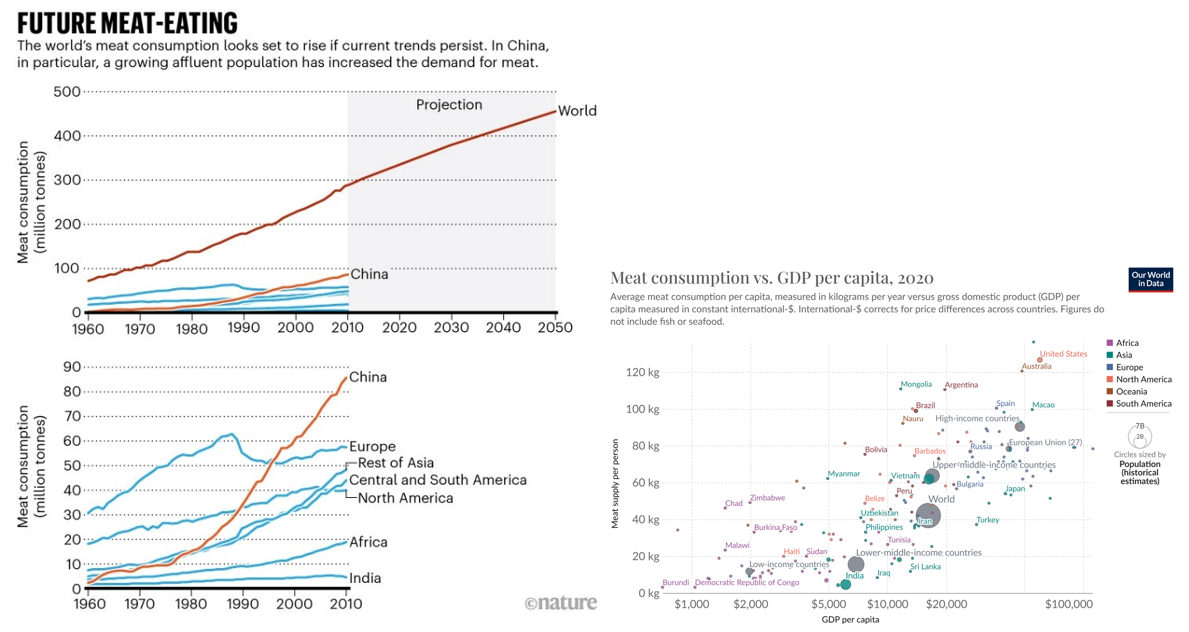
In addition to the above fundamental concerns of feeding the global population healthily without overheating the globe, other problems with current practices include ethical issues with factory farming, the risk that it causes the next pandemic, the threat to human health from bacteria possible becoming resistant to antibiotics due to farming’s usage of antibiotics, etc.
Extensive innovation has happened at the intersection of biotech and food production over the last couple decades with the major categories being:
- Replicating the biological processes that make meat taste like meat in plants (e.g. Impossible Burger)
- Fermenting GHGs into proteins
- Culturing animal cells into biologically identical meats and seafood
- Engineering crops to be more productive and / or resistant to harsh conditions
- Improving agriculture inputs like fertilizers
Following the lab work, the consumer market has grown and capital and company formation has taken hold. PitchBook estimates FoodTech’s current market size to be $73B, and investors have poured in $14B in alternative protein companies alone since 2010. Meanwhile, the traditional food companies have gotten heavily involved:
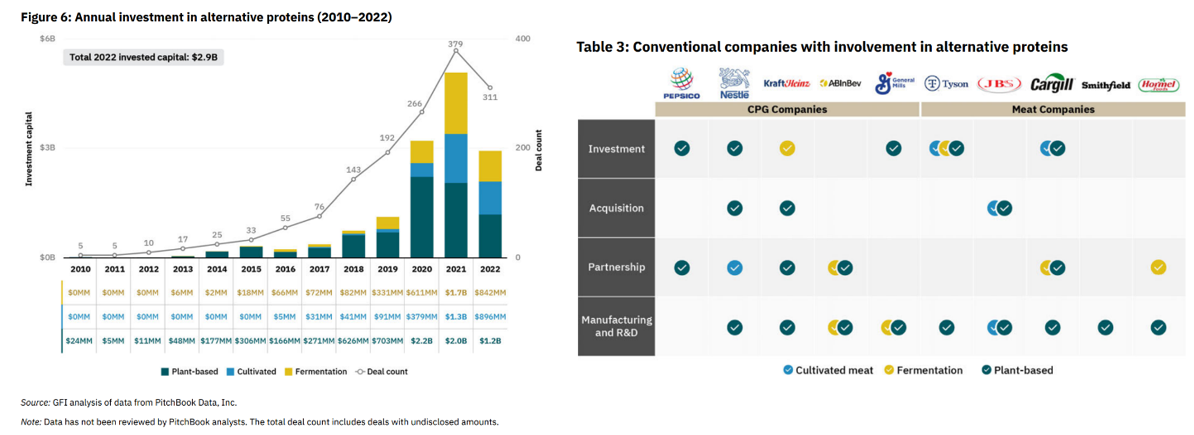
Here are some of the top cultivated and fermentation focused companies:
One note before we jump in, because I imagine the idea of unleashing technological innovation on the food we put in our bodies may make some readers uneasy, especially when we’ve been guided to eat like cavemen. The reality is that absolutely nothing we eat resembles in shape or form the original plant or animal our cavemen ancestors would’ve stumbled upon. We’ve been genetically engineering our crops and livestock to exhibit desirable traits across yield, taste, and aesthetics for millennia. Think of how alien chihuahuas are to wolves or modern corn to its ancestor Teosinte.
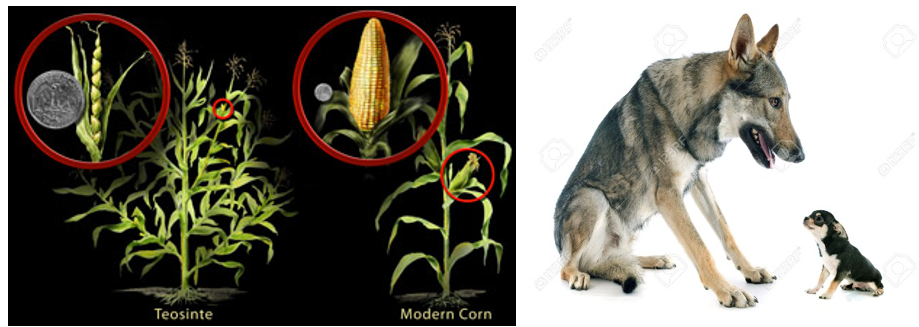
Starting in the Agricultural Revolution 12,000 years ago, gatherers would notice a crop that grew faster than others and selectively replant those seeds. Then in 1865, Gregor Mendel discovered genetic inheritance and we learned how to more intentionally breed improved traits by intervening with reproduction. Then in the 20th Century, we figured out hybridization in which we separated the parent lines of a plant, recombinant genetic engineering in which another species’ DNA is transplanted into its genome at an uncontrollable location, and radiation exposure used to randomly mutate DNA in case one variant is useful (like simulating a sped up natural selection in the lab). All these engineering techniques, along with tools from outside of biology like synthetic ammonia and robotics, have enabled us to embarrass Malthus’s prediction and feed 8 billion people. We’ve improved agricultural yields faster than population growth all the while decreasing the proportion of the population devoted to farming from 90% in 1900 to 2% today and keeping cropland area almost unchanged.
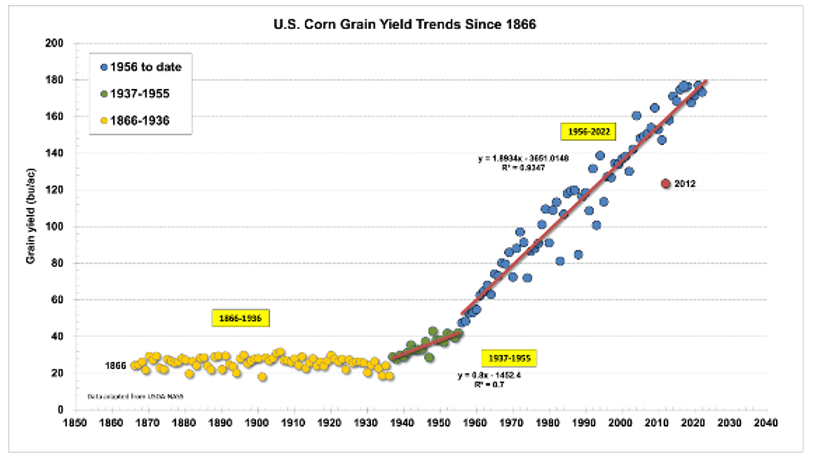
Indeed, there’s nothing wrong, unsafe, or unhealthy about “GMO” products. Every single piece of food you’ve ever put in your body is genetically modified. If you still feel uneasy, try going back to eating Teosinte and living with a wolf as your house pet.
In addition to the engineering toolkits listed above, the gene editing technology developed in the last decade enable far more precision than ever before. Instead of dousing seeds with radiation to randomly mutate its DNA or introducing an entirely different species’ DNA into it (sometimes from a different species kingdom), we can increasingly edit the species’ own genome with base-pair specific edits and deletes, like I’m writing in this Word doc. We can use this power to not just increase yield but make our crops more resistant to the hotter and more extreme weather to come or design new plants.
Meanwhile, many companies around the world are working on industrial-scale fermentation facilities to make fat and protein in environmentally and ethically positive ways. Growing our food via fermentation dates back thousands of years with staples like tofu, yogurt, and beer. We’re now doing it with fats and proteins, because while plants are highly efficient at turning carbon into carbohydrates via photosynthesis, they’re not as good at producing fats or proteins. Only a couple plants like avocados and nuts are high in healthy fats or protein. But, these crops generally entail massive deforestation and extensive water usage. Meanwhile, our dominant form of protein production comes from animals consuming many times their weight in plants before they’re ready for slaughter. The companies detailed below are looking to grow fats and proteins directly, like how plants grow carbs directly.
- Replicating animal biology in plants (e.g. Impossible Burger)
Companies like Tofurkey have been creating plant-based renditions of meats like bacon or beef for decades. But these attempts merely included taking a plant and blending it with taste additives. Few meat lovers will ever switch to a new product unless its taste is genuinely indistinguishable. The most modern efforts involve studying how the structure and chemical composition of meat at the molecular level informs its taste, texture, and aroma. Then, firms replicate that exact biological process in plants so that targeted trait is molecularly indistinguishable from the mimicked meat. For example, research at Impossible Foods identified a molecule called heme that is biosynthesized in animals’ bone marrow as what gives meat its bloody, irony flavor. They searched the plant kingdom for a gene that codes for its production and found one in soybean roots that codes for a functionally identical protein. Next, they engineered a type of yeast to express it, and then produced it at industrial scale via fermentation just like a beer brewery. When the ingredient is added to the plant-based patty, it tastes just like
Similarly, companies like New Culture identified casein as the protein that gives cheese its stringiness and melt, its funk and crumble. But it’s found only in animal milk. The company has scaled to industrial reactors, brought costs down by 80%, and secured strategic investments from industry giants ADM and CJ CheilJedang. It’s mozzarella will be served at a pizzeria in LA starting next year.
What do these things cost? On average plant-based meat is still ~2.5x times more expensive than staple meats like fresh pork and chicken, and is pricier than any major meat category except lamb. Just look at Beyond Meat’s income statement or stock chart to get a sense for the business’ current economics. With that said, remember the tricky business proportion these firms must contend with: they must make fundamentally new scientific breakthroughs and scale them to industrial capacities while competing against a product that’s been so perfectly optimized over millennia that it’s considered a literal commodity. As with all the sections below, there’s no guarantee these technologies will achieve price parity as they venture beyond the lab, but the technology deserves time to discover new breakthroughs, reach commercial scale, and optimize the processes’ efficiency as the tech matures. After all, these were merely dreams a decade ago. Moreover, it’s intuitive that a plant-based option should be able to eventually outcompete its meat analog as crops as generally a fraction of the price of meat.
An approach that may be more economical is using fungi instead of plants. They’re far closer to animals in the tree of life than plants – for instance, whereas plants photosynthesis their energy, fungi eat other biomass. As a result, their texture (dense and spongy) and taste (zin, iron, and umami) is far closer to meat than that of peas or soybeans. So, whereas plant-based alternatives require energy-intensive extrusion processes to compact them into the right texture and significant flavor additives, far less alterations are needed to replicate meat in fungi.
Companies like the Better Meat Co are able to ferment their fungi in one day from time of inoculation to harvest and sell it for less than the price of beef and eventually approach that of chicken. Additionally, it’s product has more iron than meat, protein than eggs, potassium than bananas, low in fat and cholesterol, and packed with healthy fiber. The company is partnering with the largest meat producers in the US and Canada to scale up.
What about these products’ emissions savings? As described in all the section below, the answer to this question depends massively on the assumptions made – what size bioreactor do you use (bigger ones achieve economies of scale but are harder to manage), which microbe, is it engineered, is your energy source clean, etc. With that caveat, the answer is clearly that they’re far better for the planet. This again makes intuitive sense when the crop feed necessary to grow a burger is multiple times that which is squished into a plant-based version. Indeed, third party studies commissioned for Beyond Meat and Impossible Foods found that the companies’ plant-based burgers generated roughly 90 percent less GHG emissions than their beef burger equivalent.
- Air-to-Table Dining: Converting GHGs into Proteins
Whereas the companies above ferment their key ingredients like heme and casein with agriculture crops like sugar as the feedstock for the microbes, startups and labs around the world are trying to make protein with GHGs as the feedstock, driving the products’ emissions in some cases into carbon negative. These approaches aim to replicate plants’ photosynthetic process of converting CO2 in the air into biomass later eaten by us as food. In fact, this string of research stems back to NASA, which has long dealt with the constraints of limited resources. They explored space-age goals like converting the CO2 from astronaut breath into nutritious food. As a NASA subject matter expert said, “photosynthesis can be done either by life forms or catalytically. If you are in an environment where sulfur or hydrogen are abundant, you can convert the CO2 into chains of carbon, which are basically oils.”
At least ten companies are working on doing exactly that. And, there’s room for plenty of major players, as GHGs are abundant throughout the world. The Novo Nordisk Foundation estimated that using the annual CO2 outlet from the cement industry in Egypt alone could enable protein production to help feed up to 1 billion people per year, provided we achieve our joint program targets. This would also represent a reduction in land use equivalent to an area larger than Argentina.
Some startups like Solar Foods, Air Protein, and Arkeon have found microbes that naturally cannibalize gases. For instance, Arkeon found their Methanothermobacter microbes in hot springs and feed them industry-captured CO2 from places like manufacturing plants. They do a one-step fermentation process to spit out 20 amino acids. They expect to sell the resulting nutrients to food companies for sports drinks, protein bars, and plant-based alternatives. They commissioned a pilot production plant with a 150L bioreactor system, expects a 3,000L facility within the next couple years, and then will expand to industrial-scale from there.
Other groups are genetically engineering the microbes to either efficiently consumer the GHGs or to secrete the desired output. For instance, Solar Foods collaborated with two European universities to modify GHG-loving microbes to have passages in their cell membrane large enough to funnel proteins. In this case, the group’s looking to transport β-lactoglobulin, the major protein in whey from cow’s milk. The desired secretion machinery is found natively in sugar-loving microbes, but not those that feed on gases. It’s unknown whether such foundational edits to their structure are even viable, but if so it’d enable the carbon-neutral production of milk entirely independent of agriculture and photosynthesis.
In total there at least ten companies having raised a total of ~$400M to work on turning GHGs into food. All of the approaches boast carbon intensities ranging between a 90% decrease in emissions relative to current processes to carbon negative. Time will tell if they scale in an economical way.
- Growing a Steak: Cultured Meat
TL;DR:
- Cultured Meat involves taking a clump of cells from say a cow, putting them in fermentation tanks and causing them to differentiate into muscle and fat cells using the similar molecular triggers found the animal’s tissue. Growing burgers this way results in patties that are molecularly identical to normal ones, but without the land use, ethical concerns, and potentially lower GHG emissions.
- But getting the tech to price parity will require a terrific amount of scientific and engineering innovation in culture mediums, cell lines, etc. and after all that, it still may never even approach cost parity on its own. Or, as Mosa Meat CEO said: “Is this a big endeavor with some level of uncertainty? Absolutely. Is it so unrealistic that you shouldn’t even try? Absolutely not.”
- Either way, we're about to find out. X sq ft of plants are being built right now with y capacity.
- At first it'll be mixed with cheaper plant-based alternative proteins or go after premium meats like blue fin tuna
The Economics of Making Culture Meat
- Requires three input “ingredients”:
- Starter cells, generally some type of stem cells. The suitability of the starting cells is based on their capacity for self-renewal and differentiation in an environment where other animal components, such as serum, are minimized or eliminated.
- “Growth factors” (GFs): molecules that signal to the cells how to behave. Whereas microbes multiply without any nudging, animals cells must be directed to duplicate in the first place as well as to what type of cell to differentiate into.
- Nutrients the cells need to grow and turn into the relevant tissue types, including sugars, fats, minerals, and amino acids.
Currently, GFs dominate the costs amongst the ingredients as it’s a relatively nascent product. It’s being innovated on rapidly and should come down in price. Plus, starter cells could be altered to require fewer GFs. Whereas the nutrients are basic building blocks of foods and are already produced at scale.
“Put another way, GFs carry information, which is fundamentally easier to manipulate than mass. There are unlikely to be long-term thermodynamic or biochemical limits on getting information to cells. This same is not true for amino acids. Amino acids are the fundamental building block of the proteins in meat; cells can’t be engineered to need less. In Humbird’s model, amino acids alone may constitute $7 to $8 per pound of cultivated meat (although there is by no means a consensus about this). They also have to be pure enough to avoid negatively affecting the cell culture, which adds to the price. Absent new breakthroughs, it’s possible that price floors on bulk amino acids could be a fundamental bottleneck on the price of cultivated meat.”
The combination of GFs and nutrients, called cell media, comprise over 99% of the ingredients cost7,8,9. Beyond the cell media ingredients, labor and factories add in another ~33-50%.
Tons of entrepreneurial and scientific innovation in the space have sought to drive down these costs and bring products to market. Since the first cultured burger in 2013 that cost $300K, 156 companies have been launched, almost $5B has been raised, and significant scale-up manufacturing capacity has been built. The first known pilot-scale facility opened in 2017 and now there are 18 operational facilities worldwide dedicated to producing cultivated meat or seafood with 9 more planned.
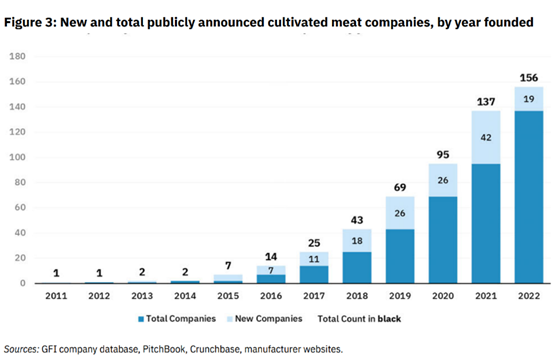
Innovation in the Space
Cell lines:
First, here’s a database of all known cell lines.
While the most accessible and thus commonly used cell lines is adult stem cells of skeletal muscle called satellite cells, their differentiation is limited to tens of doublings. Recently, interesting work has been done on infinitely differentiable cell lines like iPSCs and spontaneously immortalized cells.
A lack of readily available cell lines from relevant species and cell types has been an ongoing obstacle to scientific progress in cultivated meat. Fortunately, 2022 showed an uptick in research on cell line development. Dr. David Kaplan’s lab at Tufts University published a preprint article describing the Mack1 cell line, the first publicly available continuous myogenic (muscle) line from fish. This spontaneously immortalized Atlantic mackerel line is available for purchase by academic and nonprofit labs and can differentiate into muscle fibers as well as taking on an adipogenic-like (fat) phenotype depending on the media in which the cells are cultured. This study also provides a useful roadmap for future fish cell line development work and offers some new research tools for fish cell culture work. The Kaplan lab’s mackerel work was an offshoot of a GFI-funded project on cell line development from Atlantic salmon, and his lab receives federal funding from USDA. In a paper in NPJ Science of Food, Dr. Yusuke Tsuruwaka of Keio University and Dr. Eriko Shimada of Kyoto University and UC Davis described a fibroblast-like line established from the fin of a thread-sail filefish. One interesting feature of this cell line is its ability to be easily differentiated into a variety of lineages, including muscle and fat. This result warrants a fresh look at the transdifferentiation of fish cells, as it may indicate that finding appropriate cell lines from fish is substantially easier than we might have assumed based on results from mammals. Dr. Georgina Dowd’s group at The New Zealand Institute for Plant and Food Research also described a spontaneously immortalized myofibroblast precursor line from Australasian snapper. A paper from Professor Per Bruheim’s group at the Norwegian University of Science and Technology describes the isolation of primary muscle cells from European lobster. This is the first published report of a primary muscle cell isolation from lobster, which provides a solid starting point for future studies of cultivated crustaceans and for efforts to create immortalized lines. This paper also helps address the problem of a lack of validated antibodies for use in seafood species. Finally, Dr. Kaplan’s lab published a preprint paper describing a bovine (cow) cell line that was immortalized by expressing bovine TERT and CDK4. Academic or nonprofit groups interested in accessing the line should reach out directly to the research team.
Media:
Traditionally, most lab-scale cell culture has relied on the use of serum, a complex mixture derived from animal blood that contains growth factors, other proteins, hormones, lipids, and nutrients. While serum is effective at maintaining cell health, its use in cellular agriculture is not viable at scale due to a number of challenges including limited availability, batch-to-batch variability, and poor sustainability. Research on serum-free media advanced in 2022, with publications from Mosa Meat demonstrating serum-free bovine muscle cell differentiation and proliferation and serum-free fat cell culture protocols that can be applied across species. Researchers at the Tokyo Women’s Medical University devised a circular cell culture system in which microalgae could be used to consume the toxic metabolite ammonia while supplying nutrients to mouse muscle cells grown in serum-free conditions. They also showed that microalgae could be used as a nutrient supplement for culturing bovine cells. Formulation of cell culture media remains a challenge because each medium formulation will need to be adapted to the needs of the species and cell type being cultivated, as shown by scientists at UC Davis. To accelerate formulation optimization, the UC Davis team created an algorithm that can help researchers reduce the number of experiments needed to identify the ingredients required for cells grown under set conditions. Growth factors are currently the highest-cost ingredient within cell culture media, and several studies demonstrated substantial progress on reducing their cost. For example, a study funded by GFI and New Harvest demonstrated methods to produce and purify low-cost growth factors in bacteria. The study was also the first to test a variety of species-specific growth factors and found that some variants outperformed commercial versions. The research team’s in-house production of growth factors resulted in a 255-fold (for FGF2) or a 720-fold (for TGF-β1) lower cost than commercially available equivalents, and between a 4-fold and a 7-fold lower cost for the complete media. These cost reductions were achieved with lab-scale production, indicating the potential for much greater cost reduction when produced at a commercial scale. Other proteins commonly used in the cell culture media such as albumin and transferrin are often added in such high quantities that they can account for the majority of media cost. Researchers at Tufts University demonstrated that albumin proteins from plants such as rapeseed can functionally replace recombinant animal albumin at less than a tenth of the cost. Furthermore, researchers at the Austrian Center for Biotechnology showed that food-grade ingredients such as methylcellulose can play a stabilizing role in cell culture media similar to that of albumin, resulting in media formulations that require significantly lower quantities of costly growth factors and albumin proteins. Finally, GFI-Europe and EIT Food awarded four grants to assist in lowering media costs through strategies such as hydrolysates from microalgae, improved thermostability of growth factors, and expression in plants or cell-free systems.
Recently a low-cost serum-free medium was described for human iPSCs14. This media (B8) contains a simple mixture of basal media (glucose, amino acids, vitamins, salts, and fatty acids), minerals, and proteins, all of which can be found in animal tissue; as such, it is likely to be producible in a food-safe manner. Lab-scale B8 production was demonstrated at a cost of ~$16/L when using in-house growth-factor production. This cost could likely further be reduced with process scale-up and optimization. In contrast, media containing 20% serum or commercially available serum-free media that have been explored for satellite cells cost ~$200–500/L11,14. Because of its simplicity and cost, B8 offers a promising starting point for an effective serum-free medium that could advance cultured meat research. First, it is shown that supplementation with just one animal-free component (recombinant human albumin, expressed in rice) makes Beefy-9 media effective for BSC expansion in vitro, and that short-term growth is comparable to that in 20% FBS. Next, a protocol for passaging BSCs in Beefy-9 is established and shown to maintain cellular myogenicity in serum-free conditions. Third, cost-saving opportunities for Beefy-9 are demonstrated by lowering growth-factor concentrations without a drastic reduction in proliferation. (Source)
As I’ve discussed, amino acids could be a long-term cost bottleneck. Currently, cell culture media is made by combining each individual amino acid one by one. But this isn’t how animals get amino acids in nature. Rather, animals eat food, and then break down proteins into the constituent amino acids via digestion
Technologies that mimic this process could help lower the cost of cultivated meat in the long term. Humbird explicitly mentions soy hydrolysates as a potentially promising solution. With this technology, soy (the main source of protein for livestock) is broken down in a chemical reaction with water and added to media as a single composite ingredient. This is a novel technology with its own host of challenges, but with a longer time horizon for cultivated meat, it isn’t out of the picture (just as our highly optimized system of growing corn and soy for animal feed developed alongside industrial animal agriculture).
Another avenue to decrease the cost of amino acids is to decrease the percentage of them in the final product by focusing on fat. Since fat cells have more lipids and fewer amino acids than muscle cells, they could be cheaper to produce at large scales. This could be an important factor in the cost competitiveness of hybrid products, which can primarily get amino acids from plant-based components.
Media recycling:
Density criticisms using stoichiometry are influenced by the rate of ammonia (and less so lactate) production. A discussion on how/whether the industry may capture these metabolites and perhaps even offset costs with them is probably warranted. Modeling a scenario where ammonia is captured at the rate it is produced, or at least where its production is slowed, would be useful. In our discussions, many companies do intend to implement some form of media recycling or metabolic shunting and there is active research ongoing in the area by several companies, so greater details should be gathered from cultivated meat companies on the prospects for media recycling rather than dismissing it as unachievable.
A reduction in ammonia or lactate concentrations is not limited to externally-applied recycling systems either. A patent from Memphis Meats, for instance, describes the engineered overexpression of glutamine synthetase within cells to reduce ammonia concentrations by up to 20% while increasing the supply of the important amino acid glutamine as a byproduct of the enzymatic reaction. Other animal cells, such as those from avian species, are naturally more tolerant to ammonia concentrations due to higher expression levels of the same glutamine synthetase enzyme (Stern and Mozdziak, 2019). Scenario analyses around overcoming assumed stoichiometric limitations are thus warranted.
Improving oxygen carrying capacity:
In animal bodies, cells of course grow incredibly densely within tissues. This is only possible because of vasculature and, importantly, the presence of oxygen-carrying molecules in blood. There is precedent for using oxygen carriers in animal cell culture to increase oxygen transfer rates and thus OUR and cell density, but this is not mentioned in this analysis. Oxygen is often the rate-limiting substrate within a bioreactor, as approximately 45 times the amount of dissolved oxygen can be carried by blood versus cell culture media (Martin and Vermette 2005). For cultivated meat, the addition of recombinant heme proteins (Zhao, 2020) would be attractive not just for their oxygen carrying capacity but also their contribution to flavor, color, and cooking profiles via the Maillard reaction (Simsa et al, 2019).
Cell density and alternative processes:
Once a research group has developed appropriate cell lines and cell culture media, they can begin to scale up their bioprocess. In 2022, scientists from Believer Meats published details on their pilot-scale process, showing very high cell densities of over 100 million cells per milliliter (360 grams per liter) under continuous perfusion culture, which is the highest density reported in any cultivated meat research paper to date.
While stirred tank bioreactors are the default system ported over from therapeutics manufacturing, other bioreactor systems may offer better trade-offs for cultivated meat in the longer term. In “adherent” proliferation processes, cells are secured onto a substrate so they remain stationary, and liquid is circulated through the substrate to deliver nutrients and remove waste — similar to how blood flows in an animal’s body. Adherent processes can achieve massively higher cell densities than stirred tank systems, one of the core bottlenecks in Humbird’s analysis. It’s easy to see why: A liquid containing cells and nutrients becomes harder to mix effectively as it gets thicker and less viscous. However, if cells are kept stationary, they can be packed very tightly, as they are in natural tissue.
One reason these systems could be underexplored is that cultured meat is ultimately solving a different problem than the industries that precede it. In biopharmaceutical production, cells produce the end product, but for cultivated meat, cells are the end product. If a producer needs to extract a drug from the cell culture, it may be easier with everything loosely floating in a liquid, rather than trapped in a dense matrix of cells.
The problem with adherent systems is that it’s difficult to find comparable examples. Operationalizing and scaling up a new bioreactor system is massively more challenging when nothing is known about how cells might behave in the new environment. (link)
Microcarriers: There is no discussion of aggregate suspension culture, and the notion of using porous microcarriers that could be integrated into the final product rather than necessitating cell detachment is fairly summarily dismissed. Growth of cells on microcarriers or within other encapsulated environments could be essential to scale-up, as some data suggests that shear stress forces experienced in ultra large scale bubble column and air-lift reactors may be able to be tolerated when cells are grown on microcarriers (Li, 2020). These assumptions might make sense in typical biomedical cell culture processes, but they are being actively challenged by multiple contenders in the cultivated meat field. For example, cells can proliferate to high density within porous or degradable scaffolds, which could be used in the form of microcarriers, encapsulated tubes or spheres, or incorporated into perfusable bioreactors. As long as the scaffolds are edible, they could be used for both proliferation and maturation stages, overcoming many of the challenges regarding harvesting efficiency and other aspects of transition between these two phases. While there is still much R&D to be done, limiting the analysis to single-cell suspension systems is not reflective of the current approaches within cultivated meat companies. Additionally, it is assumed that the cells used will be immortalized adult stem cells, whereas companies are in fact pursuing a diverse set of approaches, including the use of ESCs and iPSCs. These additional approaches offer their own advantages and challenges in terms of metabolism and appropriate growth conditions.
Genetic Engineering:
Until recently, one might have thought that any sort of explicit genetic tinkering would have been a regulatory non-starter. This would make it much more difficult to achieve the metabolic efficiencies and other desirable cell traits that Humbird views as necessary (but insufficient) for cost-competitive cultivated meat. Fortunately, UPSIDE Foods’ recent FDA approval included the use of genetic engineering to make cells overexpress a particularly helpful protein as well as to immortalize one of its cell lines. This is a positive sign for the industry. It frees up companies to pursue a host of research directions to make cells more efficient and more suitable for large-scale cell culture.
“We’re taking satellite cells from cow muscle and we’re using the power of synthetic biology [CRISPR gene editing techniques] to immortalize those cells and to get those cells grow in single cell suspension, and to make sure they have all the performance characteristics necessary so we can scale them,” said March. (Source)
“The cost of animal cell culture is primarily driven by cell behavior. If you take a cell from animal muscle, it has all kinds of behaviors that don’t have anything to do with how it tastes, but which make it really expensive and complicated to grow. So they’re generally adherent cells that want to grow attached to other cells or a surface. The metabolism of the cell limits the density that will grow in a bioreactor. They’ll generally only grow in the presence of signaling molecules or expensive growth factors. But all of those behaviors can be changed using genetic engineering and synthetic biology. And so we see that as the fastest and most reliable way to solve those problems, and get to cost-effective products that can be scaled up relatively easily.” (Source)
Cell Size:
One avenue to scalability that’s unique to cultivated meat is increasing the size of each cell. Even after the number of cells in a batch is set in the proliferation phase, more mass can be added during the differentiation phase. Fat cells in particular vary by orders of magnitude in volume and could likely be grown much larger than seen in nature. Fat will likely be critical to hybrid products, since fat carries many of the important flavor compounds we associate with meat.
Humbird’s TEM underweights the potential impact of cell size, since it only considers the proliferation phase. His rationale is likely that if cell slurry can’t be produced at comparable costs to meat, then neither can fully differentiated tissue. However, if significant mass is gained during the differentiation phase, this could be a critical aspect of the total cost competitiveness of the product. Since differentiation processes differ across companies, the possibility is difficult to model.
Advancements like these over the past ten years have brought the price down precipitously, though the same trajectory shouldn’t be relied on moving forward. Current methods can make CM at a couple hundred dollars a pound. As such, in the short- to -medium term, it’ll be blended with plant-based proteins. Meatable’s CEO claims that at 10% CM, internal tasting panels indicate the hybrid is already much better than plant-based products and at 50%, it’ll indistinguishable from traditional meat. Meanwhile, the SCiFi Foods CEO believes that using synthetic biology and a blended approach, CM is commercially viable with today’s technology. And, BlueNalu’s CEO said that his approach of going after the ”Wagyu beef of the sea” should also make commercial sense today. The firm estimated it could achieve 70%+ margins on a planned 140K sq ft facility making 6 million lbs. of bluefin tuna toro a year.
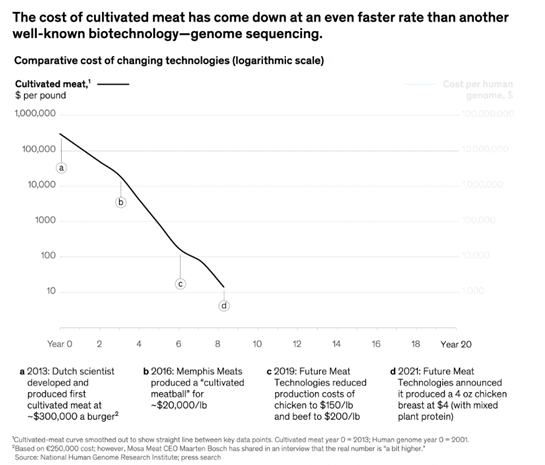
At this moment, the prospects of a non-hybrid CM product competing head on with cheaper staples like chicken have been described as “a fractal no. You see the big no, but every big no is made up of a hundred little no's.” The long-term potential of this approach, however, make some intuitive sense. The most advanced technology we currently have to make a steak is to feed cows a total of 3,600 lbs. until they reach 1,400 lbs., slaughter 300 million of them a year, and extract their organs. From that 1,400 lbs. animal, you get a couple hundred lbs. of choice cuts from an 800 lbs. usable carcass. And then you get your ½ lbs burger.
Intuitively, it feels like our technology can progress beyond that to growing the ½ lbs burger directly. The culturing approach described above seems worth exploring in part because nature itself demonstrates that our current culturing processes are remarkably inefficient. Cell densities in animal tissue are far higher than that of a traditional bioreactor, as veins are a wonderfully efficient technology for delivering nutrients to cells than stirring them around a big metal tank. And, with continued innovation in cell lines and media, combined with genetic engineering, my gut tells me we’ll be able to get there. I just have no idea how long that’ll be.
Best resources: overviews: 1,2,3; cost analysis: 1,2
- Genetic Engineering in Crops:
Researchers have been working on optimizing the latest gene-editing technologies for use in various plants, which have been more difficult to edit than animal genomes. Researchers have worked with all the latest technologies from zinc finger nucleases to CRISPR-Cas9-13 to prime editors. Consider the following examples of progress in basic science. One study last year showed a new plant prime editor several times more effective than previous methods that was used to swiftly make rice plants tolerant to herbicides in the laboratory. Another researcher demonstrated prime editing’s ability to make all 12 kinds of base substitutions and precise insertions and deletions, and its limited off-target editing. That same group released a user-friendly application to make it easier for other researchers to apply prime editing. It recommends exactly how to make the edits based on the user’s inputted parameters and intended application. It achieved 3-17x higher editing efficiency than manually designed RNAs and 2-45x compared with other web applications. Similarly, a Stanford team unveiled a comprehensive genetic toolbox program plants akin to how we program bacteria. This toolkit comprises a wide array of synthetic promoters and transcription factors, empowering researchers to control plant gene expression. These genetic components were used to make gene circuits capable of executing Boolean logic operations in a couple plant species as well as in the roots of plants, thereby managing their lateral densities.
As this technology transitions from basic science to real-world applications, many have played with its potential to increase crop yields among other potentially transformative alterations. A survey of 669 plant scientists globally suggested that maize and soybeans are expected to benefit most from CRISPR with resistance to fungus and virus to the common vehicle for implantation.
Existing research has produced several functions of CRISPR technology that could help combat food insecurity through plant breeding: herbicide resistance, drought resistance, salt soil tolerance, insect resistance, biofortification1,2,3, fungus resistance, virus resistance1,2, increased shelf life, increased fertilizer use efficiency all of which have potential to improve the sustainability of agricultural production.
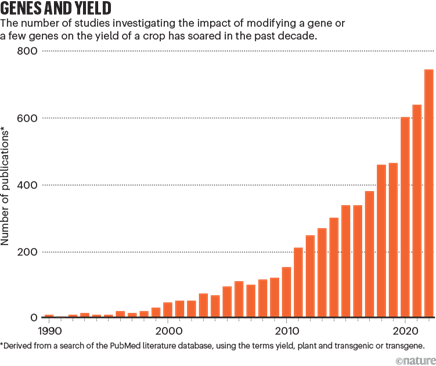
As a specific example, many groups[1] around the world are studying how to improve upon photosynthesis given that most crop plants can only convert sunlight and CO2 into plant biomass at an energy conversion efficiency of ~1% or less. This blog summarized some of the work in the field:
A couple of miles south of the University of Illinois is an area roughly the size of Central Park. Corn, soybeans, and cowpeas grow in abundance. Though it looks much the same as the surrounding fields, this is not just another farm: it’s the oldest continuously run agricultural research site in the U.S. And those plants? They’ve been engineered to grow bigger and faster, their DNA modified to speed up one of life’s most important chemical reactions: photosynthesis.
Plants use photosynthesis to harness energy from the sun, pulling carbon dioxide out of the air and turning it into sugars. Some plants are much better at this than others; the more sugar a plant makes, the bigger it grows.
Our best hope to boost crop yields is to tinker with photosynthesis directly, either by accelerating photosynthesis enzymes or by doing away with photosynthesis entirely and giving plants a more efficient alternative.
A Better Rubisco
At the University of Illinois’ South Farm, researchers from the RIPE project (Realizing Increased Photosynthetic Efficiency) are working on ways to amp up photosynthesis. They have already built plants that carry a modified form of Rubisco, a key photosynthesis enzyme that evolved 3.5 billion years ago, when carbon dioxide in the atmosphere was much higher.
Rubisco works slowly in today’s relatively low-carbon environment because it often mistakes oxygen for CO2. The enzyme captures just three molecules of carbon dioxide per second. Enzymes that break down food in our stomachs, by contrast, carry out hundreds of reactions per second.
Rubisco’s size, complexity, and unique evolutionary history make it difficult to manipulate. But a few years ago, plant engineers at Cornell University took a “fast” Rubisco from algae, loaded it into tobacco plants, and found that the plants’ photosynthesis sped up by nearly 50%. The RIPE team is using similar strategies to make bigger food crops.
When RIPE boosted photosynthesis in soybeans, “we found that the quality of the grain and the amount of protein is unchanged, but we get 20% more food,” says Stephen Long, a plant biologist and the director of RIPE, whose $45 million budget is partly funded by the Bill and Melinda Gates Foundation.
RIPE’s next target is cowpea, a legume whose best-known cultivar is black-eyed pea. Cowpea is a staple crop in food insecure regions in Sub-Saharan Africa. About 30% of Africans suffer from chronic hunger and malnutrition, according to the Food and Agriculture Organization, and that number is increasing, says Long. Hot days in the region are becoming more frequent, which hurts crop yields. Cowpea is a leading source of protein for over 200 million people, but Nigerian farmers, its main producers, cannot grow enough to meet demands.
Unfortunately, boosting cowpea and rice yields by modifying Rubisco directly will take a really long time. For a single experiment, it used to take three months to modify the enzyme, insert it into plants, and wait for them to grow, according to Maureen Hanson, a plant geneticist at Cornell. That slow pace handicapped experiments because researchers can only test a few versions of engineered Rubisco each year. Hanson published a study in late 2020 that could sharply cut that time. She took Rubisco from a plant and placed it inside of E. coli bacteria, which double in population every thirty minutes or so. “Now we can say, ‘Oh, let’s change that amino acid there,’ and see what happens” in a few days, rather than months, she says.
Other scientists are less concerned with engineering plant Rubiscos because they think that a better version of the enzyme is already hiding in nature — no engineering required.
Israeli molecular biologist, Ron Milo, searched genetic databases and found 33,000 different types of Rubiscos in thousands of plant and microbial species. His team at the Weizmann Institute of Science studied a subset of them in test tubes and watched to see which ones worked fastest. Milo called the experiment “the Rubisco-lympics.”
Back in June 2020, his team found a Rubisco from cyanobacteria, a photosynthetic organism, that worked six times faster than a typical plant version. Milo’s team is now inserting those enzymes into crops to see whether it makes them larger.
Other researchers, though, see Rubisco as a dead end entirely. “Rubisco has evolved over billions of years,” says German bioengineer Tobias Erb of the Max Planck Institute for Terrestrial Microbiology. “You don't teach this old enzyme new tricks.”
Go Synthetic
In Asia, a hectare of land today feeds 27 people. By 2050, that same land will need to feed 43 people. Traditional rice breeding has only increased yields by about 1% year-over-year.
The C4 Rice Project — a global consortium of researchers from 7 institutions in 5 countries — aims to boost yields by converting rice’s photosynthesis from its native C3 to another type, called C4.
Most C4 plants (like maize) are much more efficient because they use two separate compartments for photosynthesis. As CO2 comes into the cell, it is first fixed by pep carboxylase, an enzyme that doesn’t inadvertently bind to oxygen. The product of this reaction is then shuttled to a different cell, where Rubisco lies in wait. C4 plants are basically CO2 concentrators — less oxygen gets to Rubisco, and photosynthesis is faster as a result.
Back in October 2020, the C4 team took five genes from maize and ported them into rice. The added genes didn’t boost crop yields much, but it was an early step that indicated a C3 to C4 conversion might be possible.
And just a few days ago, scientists discovered that Portulaca plants perform C4 and Crassulacean acid metabolism (CAM, another carbon fixation pathway) photosynthesis in the same cell, thus providing evidence that the hard part of the C4 Rice project — namely, spatially isolating the different steps in photosynthesis — might not be needed after all.
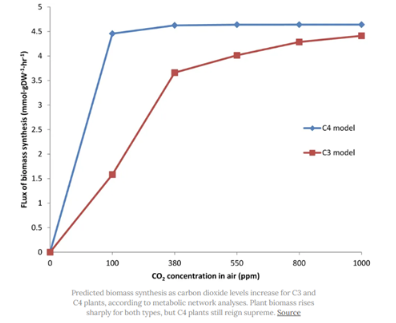
If RIPE and the C4 Rice projects fail (and they might), other researchers will be ready with alternatives.
In the past year, both Milo and Erb have entirely rebuilt the chemical process for photosynthesis in their labs. In 2016, Erb created an artificial photosynthesis system, called the CETCH cycle, using 17 different enzymes from various plants and microbes, including three enzymes that his research team designed from scratch. Each “designer” enzyme was 3D-modelled on a computer, created in the laboratory, and tweaked over a four-year period before the system worked as expected.
When the enzymes were added to a test tube, the artificial photosynthesis was about five times faster than natural versions. But Erb, at the time, couldn’t get the enzymes to work in living cells: “It's like building an engine and then trying to put that into an existing car,” he says.
A couple years ago, in Science, Erb’s group also reported an artificial chloroplast — made from spinach chloroplast membranes and their CETCH enzymes — that uses sunlight to convert CO2 to sugar. And last year, Erb’s team built a rationally-engineered enzyme that rivals the efficiency of natural CO2-fixing enzymes. Based on computer modeling, they predict that the enzyme could boost the efficiency of synthetic CO2 fixation by 150%.
The following review surveys the work in genetic engineering more broadly:
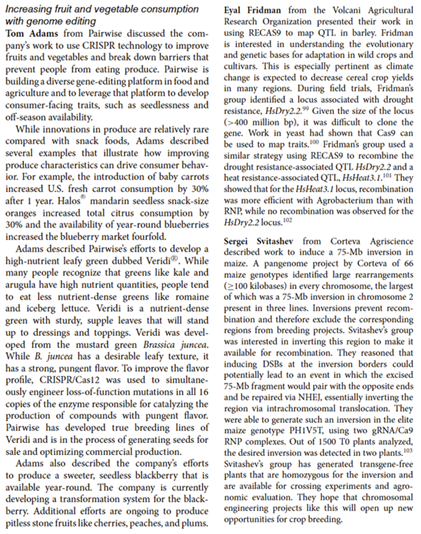
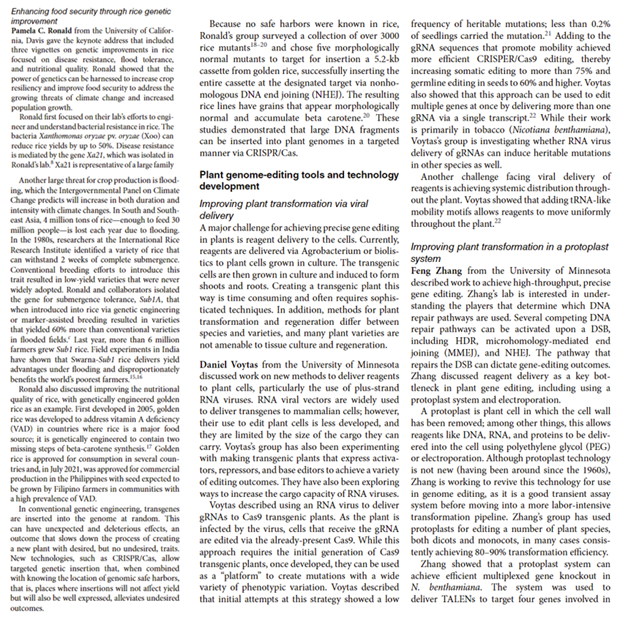
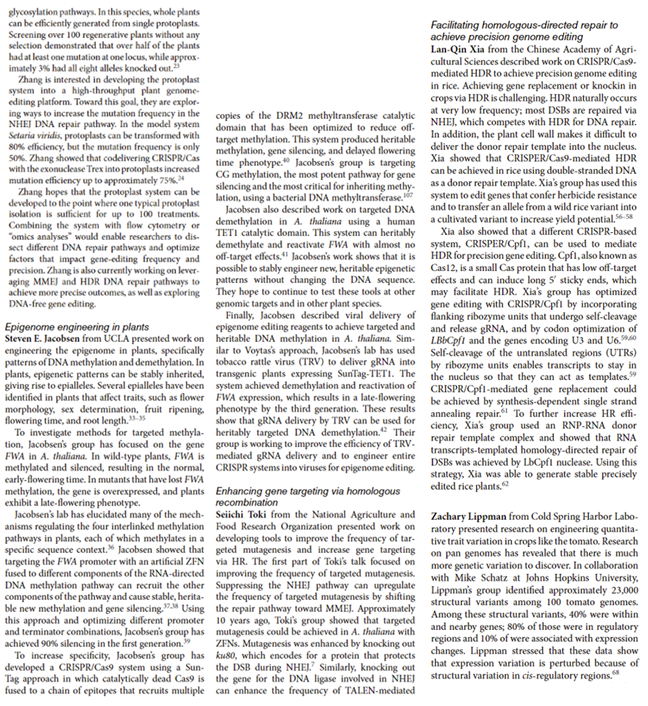
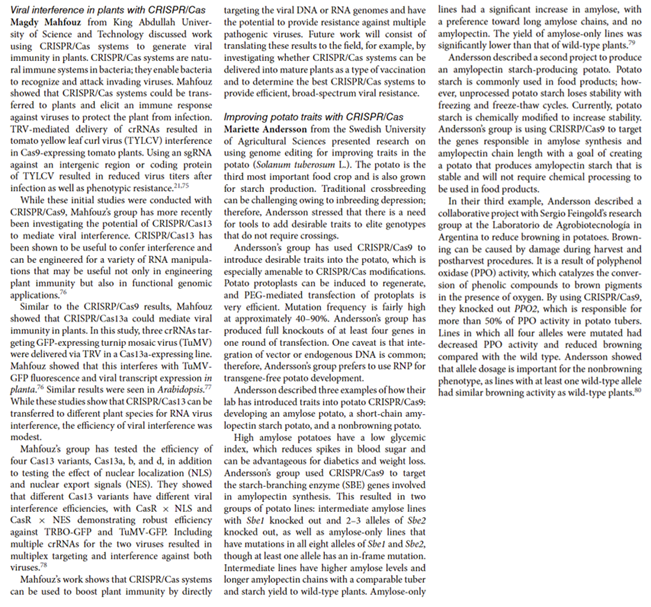
With all this said, this technology remains immature, so one should be careful to not let the cart get in front of the horse. Here are some of the biggest scientific question-marks remaining:
First, before any manipulation can be performed on the genome, the specific gene responsible for the intended function must be identified to enable precise editing. Despite the efforts conducted to sequence the genomes of many relevant plant species, there is still insufficient knowledge on the function of sequenced genes within the plants’ genome, which impedes efforts in precision editing to produce intended effects (Haque et al., 2018; Adhikari and Poudel, 2020). Fortunately, by conducting Genome-Wide Association Studies (GWAS), gene functions can be effectively predicted with accuracy, which can drive further research on necessary manipulations in plants. For instance, Zheng et al. (2019) discovered the genes OsC1 and OsRb that are involved in regulating anthocyanins in rice leaf. This enabled Hu et al. (2020) to further use the CRISPR/Cas9 technology in manipulating anthocyanin levels in rice. A similar approach was also undertaken to study the RDP1 gene of A. thaliana (Tsuchimatsu et al., 2020). Just as how GWAS can propel CRISPR/Cas9 plant editing, CRISPR/Cas9 technology is also used as an alternative method for cross population validation (Alseekh et al., 2021), such as to validate GWAS findings in rice (Oryza sativa) (Lu et al., 2017; Meng et al., 2017) and maize (Zea mays) (Liu et al., 2020). This provides an insight into the importance of establishing the causal relationships and interactions between genes that can further drive the development of CRISPR/Cas9 technology in plants (Yin et al., 2017)
As for regulatory hurdles, the US and EU differs greatly. In the EU, gene-edited plants are regulated the same way as GMO products despite not introducing a new species’ genome. This will stifle research and company formation in the space. In comparison, the US Department of Agriculture affirmed the technology’s limited risk by explicitly ruling out regulation of genome-edited plants, provided its production does not involve plant pests. Additionally, the USDA passed the first genome-edit crop in 2016 and has continuously funded research into gene editing a variety of crops.1,2,3
The biggest obstacle of all isn’t technical or regulatory. Scientists across the board view consumer perception as the biggest barrier to CRISPR adoption. Hopefully we can be reminded every once in a while of chihuahuas and Teosinte.
- Improving Agriculture Inputs:
A final area of research involves making inputs like ammonia or nitrogen fertilizer with bio-based methods. One area of particular interest is de-carbonizing the production of nitrogen fertilizer or reducing our need for it. We currently make ammonia via the Haber-Bosch process, a discovery from the early 20th Century without which 4 billion people wouldn’t have enough food. It also emits 1% of all GHGs and consumes 2% of the world’s electricity production. Moreover, half of all nitrogen applied to cropland is lost due to over-application or application at the wrong time.
Two prominent startups addressing the challenge, Pivot Bio and Joyn Bio, engineered microbes that adhere directly to the roots of crops, fix nitrogen from the air, and feed it to the crops. Bacteria naturally do so for soybeans and other legumes but are not compatible with cereal crops like corn, wheat, or rice. These startups’ engineered bacteria turn on the necessary genes to facilitate that process in cereal crops’ roots. While Joyn which is a joint venture between Gingko and Bayer is still a few years from commercialization, Pivot has raised $700M and reached meaningful scale. Its corn fertilizer is used on 5 million acres, rising from 250 thousand three years prior and generating the firm $100M in revenue. The product reduces the need for chemical fertilizer by 25% without impacting yield. The savings on reduced fertilizer usage simultaneously increased farmer profit by $16/acre and avoided 226,000 tons of carbon emissions. The company is building a 48K sq ft plant to manufacture its products for corn and wheat as well as a 110K sq ft distribution center.
Other groups are engineering plants themselves to be able to engage in this symbiotic relationship with nitrogen-fixing bacteria, again something only found in legumes in nature. 1,1,1
Another company called Indigo, spun out of Flagship, provides plant microbiome agricultural services designed to increase crop yield. The company's plant microbiome agricultural services use a database of genomic microbe information to predict which microbes are most beneficial to the health of the crops and apply these microbes as seed coating. Other companies are developing biological rather than chemical pesticides, new vaccines for crops (including RNA vaccines that selectively turn on or off genes in plants, pests, or pathogens), biosensors to optimize inputs like irrigation, and more. See this report for a broader survey of the industry.
A final note: the cutting-edge efforts above must be coupled with modernizing the farming techniques of the remaining places in the world where they’ve yet to be adopted like Africa and to find political, behavioral, and technological solutions for the West to stop wasting half our food output.
Conclusion
Not only can these products be far more environmentally across multiple dimensions (GHG emissions, land use, water use) and improve animal welfare, but one must also view the future of our food supply as a national security issue. Assuming that at some point we’ll need to transition away from consuming ever-more animal meat, then the nation must insist on industrializing the products and processes that the US has led the innovation of. We are at a juncture similar to solar panels years ago. The US’s natural science labs invented all the chemistry necessary to make them but then allowed their manufacturing to flood overseas to Asia. In particular, China controls the 80%+ of many components in the process, thanks in part to heavy subsidies. We’ve finally woken up to and are seeking to address1 the vulnerability of having one of your chief enemies control you access to the next form of energy production, likely spurred by the fall out of energy markets in Europe after Russia’s invasion. Such a vulnerability with food feels even more concerning, especially as food becomes a high-tech good, not something you simply pull out of the ground.
Second, some of the above technologies may need government support in the form of low interest loans given to clean-tech companies in other industries to bridge the gap between lab scale and outcompeting a dirt-cheap commodity on price and taste. Moreover, it’s not as though it’s a level playing field to begin with. These commodities are dirt cheap in part because they’re heavily subsidized. The Biden administration alone has spent $1.5B to increase slaughter capacity1,2. There’s also federal excess production purchase programs. A recent example is the USDA stepping in with $470M1. A third way meat production is subsidized is via the subsidization of its input, crops (e.g. insurance programs, guaranteed purchases). Finally, they do not pay for the negative environmental externalities they create, the risk that factory farms will cause next pandemic, or animal welfare. All this isn’t to argue that these programs should be reversed, but to point out that the meat and produce industries feed from the hands of the US government. An infant industry deserves at the very least a level playing field.
Read next section: sustainable fuels & chemicals
[1] MIT, UC Riverside, Lawrence Berkeley Lab, Max Planck Gesellschaft (1,2,3)